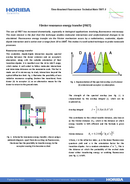
TCSPC stands for time-correlated single photon counting. It is a method of using the timing of a pulsed excitation source, like a laser or LED, with the timing of the arrival of single photons on a detector to reconstruct the lifetime decay over many events (repetition of pulses and photons detected). This technique is based on the fact that the probability of detecting a single photon at time, t after an exciting pulse is proportional to the fluorescence intensity at time t.
The repetition of a laser or LED pulsed at relatively high repetition rate (10 kHz to 100 MHz) is synchronized with the time the next photon arrives at a detector (i.e. PMT). Timing electronics in the form of a time-to-digital converter or time-to-amplitude converter (TAC) are used to record these events in succession, until sufficient statistics are collected to reconstruct the decay. The decay is then fit to an exponential function to model the lifetime decay (t). TCSPC is typically used to measure fluorescence lifetimes from the picosecond to microsecond time scale.
Using the kinetic TCSPC mode, individual measurements in as little as 1 ms can be made and up to 10,000 measurements can be seamlessly acquired. As long as a fluorescence lifetime change occurs, then this approach, rather than intensity, can be used to follow a kinetic process.
Obviously, a sufficient number of photons are needed to be able to analyze the data. This can be enhanced by using a very high repetition rate, but the lifetime and time range needs to be considered, so as not to re-excite the sample before it has completely decayed. The lifetime data can then be used to construct kinetic traces for the process.
Förster Resonance Energy Transfer
If you can measure fluorescence, you can measure FRET (Förster Resonance Energy Transfer). FRET is the interpretation of the measurement result, rather than a measurement technique. FRET occurs when the emission of a donor molecule overlaps with the absorption of an acceptor molecule. When the two are close enough, they undergo a dipole-dipole interaction and energy is transferred. The distance at which there is 50% transfer energy is called the Förster distance and this value is typically known for common FRET pairs. By measuring the change of fluorescence intensity or lifetime of the donor molecule in the presence of the acceptor, the FRET efficiency and therefore the distance between the two can be found. FRET can be measured using either fluorescence spectra (intensities) or fluorescence lifetimes.
Förster Resonance Energy Transfer
Several measurements are needed; ID, the intensity (or tD, the lifetime) measured at emission peak of donor alone, IDA, the intensity (or tDA, the lifetime) measured at emission peak of donor in presence of an acceptor, IA, the intensity (or tA, the lifetime) measured at emission peak of donor with the presence of an acceptor but not the donor and IB, the intensity (or tB, the lifetime) measured at emission peak of donor using blank solution (i.e. buffer only). From these, the efficiency, E, can then be used along with the Förster distance (R0) to calculate R, the distance between the donor and acceptor molecules being measured. See the equations in the figure above.
FRET principle
Static and dynamic quenching
Quenching refers to the reduction in fluorescence emission, i.e., an increase in the non-radiative decay rate (knr). Quenching can be divided into “static” and “dynamic” forms. In both cases, the emission intensity deceases, but only in dynamic quenching is there a change in the fluorescence lifetime. Note: If the kinetics follow Stern-Volmer kinetics and an average lifetime is used, then this should be calculated using the intensity average.
Photobleaching of sample obvious in intensity measurement
The effect of photobleaching will increase the measurement run time as it effectively reduces the number of fluorophores emitting (i.e., acts like a decrease in concentration), and reduces the emission intensity. The lifetime will be unaffected.
TRES of 2-naphthol and 2-naptholate A
Time-resolved emission spectral measurement is a technique that measures the fluorescence decay at incremental wavelengths across the emission spectrum of a sample. A 3D plot of intensity versus time versus wavelength is obtained. By looking at this 3D data set in the direction of spectra at different times instead of decays at different wavelengths, the time-resolved emission spectrum can be measured. If a sample contains multiple emitters with overlapping spectra but different lifetimes, the individual spectra of these components can be separated using TRES.
For example, 2-naphthol becomes ionized to make 2-naphtholate in the excited state. (Koti, 2001). The emission spectrum in the steady state shows two peaks, indicating both species are present. Measuring the lifetime at incremental wavelengths across the emission spectrum show very different decay rates at each emission peak. By fitting the decays, one can see how the component lifetimes and/or amplitudes change at different emission wavelengths.
For 2-naphthol, the emission peak of 2-naphthol at 354 nm has a different lifetime than that of 2-naptholate, which has an emission peak around 414 nm. The 2-state model of ionized and non-ionized 2-naphthol is clearly shown in the TRES. Two time constants represent the different decay times for 2-naphthol (3.4 ns dominant at 357 nm) and 2-naphtholate (9.4 ns dominant at 414 nm). The data shown below was measured on a FluoroMax-4 with FluoroHub TCSPC electronics and a NanoLED-280 excitation source operating at 1 MHz.
Another use of TRES is the measurement of solvent reorientation time constants. (Horng, 1995). By looking at a single fluorophore and how the emission spectrum shifts over time, the peak energy versus time can be plotted and fit to get a time constant. The shift of the spectrum in this case can be due to solvent molecules reorienting their dipole moments in response to an excited state dipole moment of the fluorophore. By fitting the peak energy of the emission spectrum over time, the reorientation time constant(s) of the solvent molecules can be obtained. (Horng, 1995).
Yes. There are a couple of ways to do this, depending on the sample. A kinetic TCSPC measurement can be performed to monitor binding if the lifetime changes during the binding process. Time-resolved anisotropy can also be employed, as binding will affect the rotational correlation time. Because this is due to the change in effective size of the molecule, the rotational correlation time is proportional to the effective volume of the molecule.
The applications of phosphorescence measurements
In one example, a delay is used after the lamp has flashed to measure the phosphorescence spectrum. Without a delay, both the short-lived fluorescence from the peptide in this sample as well as the longer-lived phosphorescence of the terbium can be observed.
The applications of phosphorescence measurements
By varying the delay, one can selectively detect species with longer-lived phosphorescence separate from background fluorescence in the same sample.
The composition of lanthanides in glass materials can be studied using time-resolved phosphorescence decays. Here is data from the study of erbium content in different glasses using this method. The lifetime of erbium can vary with different types of glass and processes used to make the glass.
PL decay of europium chloride in water
The boxcar technique, or boxcar averaging, is a method to measure a phosphorescence or long-lived fluorescence decay by integrating at fixed integration windows across the decay time of the signal.
A pulsed source, such as a xenon flash lamp, is flashed and a delay is set after the pulse (ideally a time when the lamp flash is completed). The detector measures an integration window repeatedly to get a statistical average of the intensity at that window after the flash. Then, the integration window is moved incrementally across the decay to longer decay times. In this way, a decay is produced and can be fit with an exponential decay to get the lifetime by the inverse of the decay rate. The boxcar technique can be very slow, especially with long-lifetime decays and weak emitters. However, depending on the width of the xenon pulse, lifetimes between 10 μs and seconds can be resolved in a relatively low cost way, using a tunable light source.
An example application of SSTD: room temperature phosphorescence (RTP) of Nase T1 tryptophan
SSTD stands for single shot transient digitizer. The SSTD technique utilizes a pulsed light source, either a pulsed laser or a xenon flash lamp to acquire an entire phosphorescence decay curve from each flash of the pulsed source. After each pulse, the decay is captured and digitized in real time with a PMT and a transient digitizer. A rapid signal averaging can easily be achieved, since a complete decay is measured after each shot. The time-resolved spectra can easily be measured by numerical integration of the decay signal within the user defined time range and scanning a monochromator. This allows for discriminating spectra based on the lifetime of the respective excited state.
Phenanthrene fluorescence and phosphorescence spectra measured while increasing the delay time at 2 μs increments for signal integration.
Fluorescence emission happens on the picosecond to nanosecond time scale, while phosphorescence that is measured with SSTD occurs on the microsecond to second time scale. By varying the temporal position and the width of the signal etection gate, you can selectively detect fluorescence and phosphorescence spectra, as attested by phenanthrene spectra on the accompanying figure. Here, the emission of phenanthrene in a frozen glass was measured with a gradually increased time delay of the detection gate to diminish the contribution of fluorescence.
Discrimination between strong fluorescence and weak room temperature phosphorescence from RNase T1 tryptophan.
An example application of SSTD can be seen in the case of room temperature phosphorescence (RTP) of Nase T1 tryptophan. Here, the signal was extracted by gating out the overwhelming tryptophan fluorescence, which would have been extremely difficult to do using a continuous excitation source. The phosphorescence decay of the very weak emission was also measured on the same instrument by using the Single Shot Transient Digitizer (SSTD) function (HORIBA PTI QuantaMaster Series, 2017).
Schematic diagram of Strobe
The stroboscopic optical boxcar technique is also referred to as the Strobe technique. It is a time domain, pulsed technique that flashes a pulsed light source and then activates a PMT, by sweeping a very short temporal duration high-voltage pulse down the dynode chain of the PMT. Subsequently, these flashes are repeated and through the use of a delay gate generator, the intensity is measured at a different time after the flash, to construct a decay curve. This method then uses multiple flashes and averaging to improve signal to noise of the decay.
The Strobe technique is an analog technique that is inherently less sensitive than TCSPC and does not enjoy true Poisson statistics as does TCSPC. However, it has the benefit of providing decays from about 150 ps to seconds with low repetition rate sources such as a laser diodes, LEDs, tunable Q-switched OPO, or nitrogen/dye lasers. The Strobe technique can measure ns time-resolved spectra directly by fixing the position of the delay gate and scanning the emission monochromator.
One of the advantages of the Strobe is that it can collect decay in both linear and non-linear (i.e. arithmetic progression and logarithmic) time scales. The latter greatly aids in resolving multi-exponential decays where lifetimes can differ by orders of magnitude. Another advantage of the Strobe technique is that it can work with low repetition rate tunable lasers, such as Q-switched/OPO or nitrogen/dye, which cannot be used with TCSPC. It can also work with LEDs and laser diodes operating up to about 25 kHz.
Complex decay from ZnO solid sample.
An example of lifetime measured with strobe is the lifetime decay and distribution analysis of ZnO slides as shown in the following figure.
Fluorescence up conversion is a 2-photon process, whereas a sample is excited by two contemporaneous photons arriving simultaneously in the near-infrared wavelength region and fluorescence is emitted at higher energy (lower wavelength) in the visible region of the spectrum.
Fluorescence up conversion accessory for the Fluorolog-3 (left) and a QuantaMaster (right).
While the excitation power needed will depend on the sample in question, up-conversion typically requires a higher photon flux excitation source such as a laser. Because of this, standard TCSPC sources may not have the required flux to perform up-conversion measurements. Lasers that output 980 nm can be mounted directly onto a sample compartment to excite samples directly for measurement of up-conversion spectra, lifetimes, or even quantum yields. Q-switched OPO lasers with efficient NIR output can also be used for the measurement of fluorescence up-conversion.
Steady state upconversion emission of Er3+ doped nanoparticles.
Molecules that absorb light in the NIR and can be detected or even imaged in the visible range are useful. This is because high-energy UV excitation sources tend to photobleach or cause photo-damage in biological samples. NIR sources are exciting at lower energy and typically do not have this issue. Molecules that exhibit fluorescence up-conversion include lanthanides, semiconductor nanoparticles and quantum dots. The same 980nm DPSS laser can be pulsed by TTL pulses and used for the PL up-conversion lifetime measurements, either with the MCS function of the TCSPC board or SSTD technique.
Although fluorescence (and phosphorescence) has a large range of applications, there are two major areas of research where the use of this phenomenon excels:
In any application where steady state fluorescence is found, it can be advantageous to employ lifetime methods to obtain information. Some of these applications and fluorescence techniques that can be employed in their study are illustrated below.
STRUCTURE/CONFORMATION | SIZE/MOBILITY | FUNCTION |
Monitor
Using techniques such as
| Monitor
Using techniques such as
| Monitor
Using techniques such as
|
SEMICONDUCTORS | GLASSES & POLYMERS | NANOPARTICLAS INCLUDING QUANTUM DOTS |
Monitor
Using techniques such as
Applications
| Monitor
Using techniques such as
Applications
| Monitor
Using techniques such as
Applications
|
Do you have any questions or requests? Use this form to contact our specialists.